Summary
Ultrasound imaging is a clinically approved and routinely used technology capable of visualizing anatomy of soft tissues. At the same time, this method lacks characterization of tissue composition and has poor contrast because the ultrasound impedances of most soft tissues vary insignificantly. Laser-based photoacoustic imaging is an ultrasound-friendly imaging modality which utilizes optical contrast and can reveal pathologies such as lesions or lipid-rich plagues.1,2 However, both these methods could not provide any information about mechanical properties of soft tissues, such as Young’s modulus, which are also indicative of pathologies.3 The mechanical properties of tissues can be reconstructed by an elasticity imaging which is also ultrasound-friendly and can be both static, when the tissue is mechanically deformed during the imaging, and dynamic, when shear wave is generated by acoustic radiation force (ARF) and the speed of the wave propagation is used to reconstruct shear modulus.
To combine all three imaging modalities and enable combined ultrasound, laser-based photoacoustic and elasticity (CLUE) imaging, an efficient probe comprising an ultrasound array and light-delivery system is required. However, there are a few complications. First, high-resolution ultrasound imaging and detection of small displacement of tissues caused by propagation of shear wave require high-frequency ultrasound. On the contrary, photoacoustic transients is expected to be in low-frequency range.4 Also, generation of shear wave using ARF is more efficient at low frequencies. The ultrasound array should be, therefore, high-frequency and low-frequency simultaneously. Second, SNR of photoacoustic imaging increases with the light fluence delivered to the imaged area. Overall, the quality of photoacoustic imaging improves when light beam and ultrasound beam coincide in imaging plane. Obviously, the transducer cannot emit light. Finally, the design of the CLUE probe should be convenient to handle and use.
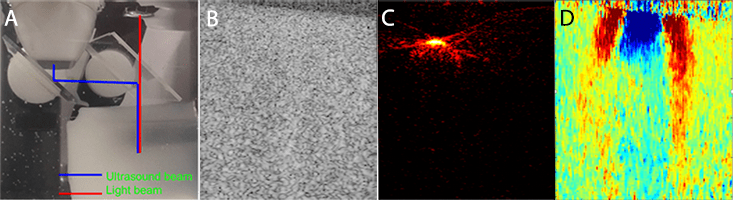
All these problems could be resolved by using two ultrasound arrays – one low- and one high-frequency – and a 1-to-1 leg light delivery system positioned between. Feasibility of this design was initially tested using one ultrasound array and an optical bundle with one output light bar. Figure 1 shows a photograph of the CLUE probe conceptual design and initial phantom results. The ultrasound array will be installed in parallel to the light delivery system. Light will be delivered through a transparent ultrasound reflector, it could be a prism or glass plate. Ultrasound beam will be twice reflected such that it coincides with light beam.
The phantom was made of 8% gelatin with 1-cm-diameter inclusion made of 12% gelatin. 200-300 mesh silica particles with concentration of 2% for phantom and 1.8% for inclusion were used as ultrasound scatterers. Graphite particles with 0.2% concentration was used to provide optical contrast and to match concentrations of ultrasound scatterers in inclusion and phantom. As expected, ultrasound image does not show both location and size of the inclusion. Photoacoustic image depicts the location and shape of upper part of the inclusion; however, light does not penetrate deeper due to high light absorption coefficient of the graphite filament. SWEI allows to estimate both size of the inclusion and its stiffness. Indeed, the shear wave speeds up into inclusion which indicates elevated shear modulus compared to the phantom.
Overall, the experimental results demonstrate that both ultrasound and SWE imaging is feasible despite the dual reflection of ultrasound waves. This is especially important for SWEI. In addition, photoacoustic imaging has enough contrast and becomes free from photoacoustic artifacts caused by light, reflected and scattered from the imaged tissue.5 The probe can be compact and easy handled. All transducer wires and optical bundle can be integrated into a single hose for better handling.
Future work includes investigation of material and shape for the ultrasound reflector and for central prism, development of light delivery system for better irradiation of the imaged area, case design for handle the probe conveniently, development of software to enable real-time imaging at different wavelength and to integrate the probe with existing imaging systems.
References
- K.S. Valluru, J.K. Willmann, “Clinical photoacoustic imaging of cancer,” Ultrasonography 35, pp. 267-280, 2016 ^
- A.B. Karpiouk, B. Wang, J. Amirian, R.W> Smalling, and S.Y. Emelianov, “Feasibility of in vivo intravascular photoacoustic imaging using integrated ultrasound and photoacoustic imaging catheter,” J. Biomed. Opt. 17(9), 096008, 2012 ^
- A. P. Sarvazyan et al., “Shear wave elasticity imaging: a new ultrasounic technology of medical diagnostics,” Ultrasound Med. Biol. 24, pp. 1419–1435, 1998. ^
- G. J. Diebold and T. Sun, “Properties of photoacoustic waves in one, two and three dimensions,” Acustica 80, pp. 339–351, 1994. ^
- Yuanyuan Bai, Bing Cong, Xiaojing Gong, Liang Song, Chengbo Liu, “Compact and low-cost handheld quasibright-field linear-array probe design in photoacoustic computed tomography,” J. Biomed. Opt. 23(12), 121606, 2018. ^